Introduction
Caenorhabditis elegans, commonly shortened as C. elegans, is a non-parasitic nematode which, for its peculiar proprieties, is widely used as a model organism in biology. Indeed C. elegans, since Sydney Brenner’s pioneering studies in the 1970s (S. Brenner, 1974), has been the subject of several investigations, especially focused on its nervous system as it is one of the simplest organism having one. This interest led the C. elegans to be the first multicellular organism to have its whole genome sequenced. The vast knowledge and its unique transparency features make C. elegans a choice of election for biologist in the field of live cell imaging.
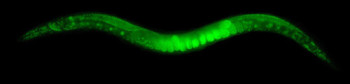
The most important prerequisite to fulfill in order to achieve high-resolution, live cell videos and images of C. elegans is the immobilization of the animal. Indeed, contingent movements can give raise to artifacts or out-of focus images, making the whole observation useless. Immobilization methods can be divided under two general approaches: chemical techniques and physical techniques.
The first category embraces all methods which achieve immobilization by means of any chemical compounds, such as metal ions or antibodies. Although these techniques can provide good results in terms of immobilization, most of them appear to affect the cells adversely, even using really low concentrations. The physical techniques, on the other hand, generally depend on alterations of viscosity of the medium through or on some means of capturing and holding the worm. A more detailed and exhaustive review of these methods can be found in Aufderheide (2008).
Concerning C. elegans, the most common techniques found in the literature to obtain the immobilization of the worms rely on the usage of glue or anesthetic drugs. Although these methods are well-established, they can potentially affect the sample inducting physiological modifications or prevent future manipulation. Furthermore, they are low-throughput techniques as it is rather difficult and time-consuming to immobilize a large number of nematodes with these methods. Even using a more recent and sophisticated mean to obtain C elegans immobilization, such as polystyrene nanoparticles (Kim et al., 2013), while it is easier to set up has its drawbacks. The most restrictive one is that immobilization, in this case, depends on worm growth stages and thus requires a presorting of worm’s population.
Microfluidics can be the answer to this and other issues regarding the study of C. elegans, as it not only allows controlled flows and drug delivery to the sample, but it also provides a platform for animal easy manipulation and handling, which is otherwise manual and time-consuming. Additionally, the use of glues and anesthetics for studies that require worm immobilization is avoided.
Object of this short review is indeed the description of the most common microfluidic device and techniques to achieve C. elegans immobilization.
Ultra fast temperature shift device for in vitro experiments under microscopy
C. elegans immobilization via microfluidics for live cell imaging
In recent years many methods and devices in microfluidics have been developed in order to obtain on-chip worm immobilization. The most notable have achieved immobilization by cooling (K. Chung et al., 2008), compression or restriction (T. V. Chokshi et al., 2009; S. E. Hulme et al., 2007), CO2 (T. V. Chokshi et al., 2009), and gelation of the surrounding fluid (J. Krajniak and Lu, 2010). In the following, these techniques will be briefly presented.
1. Microfluidic temperature-induced immobilization of C. elegans
This method, developed by Kwanghun Chung (K. Chung et al., 2008) addresses the key challenge of in vivo microscopy, phenotyping and screening, of having a hardware capable of handling worms in a way compatible with standard readouts and that allows automation on observation and manipulation of the sample.
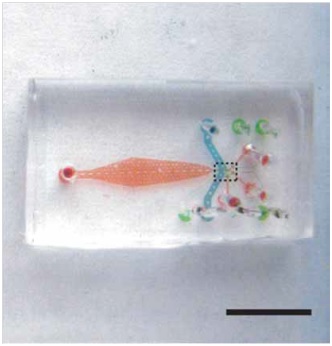
In this technique, it is employed a polydimethylsiloxane (PDMS) microfluidic chip with built-in valves to control a suspension of nematodes (Fig. 1.1). The crucial feature of this device lies on the integration of several key design features that ensure experiments with high-throughput. Additionally, an algorithm can be employed in order to automate the entire process of image acquisition, analysis and sorting, which allows the system to operate without human intervention. Chung’s protocol rely on 4 steps (Fig. 1.2): worms are first loaded into the microfluidic device by a constant pressure-driven flow, then the sample is immobilized reversibly by cooling and thus scanned by high-resolution microscope. Subsequently, phenotyping and sorting take place and the obtained videos/images are stored for further analysis.
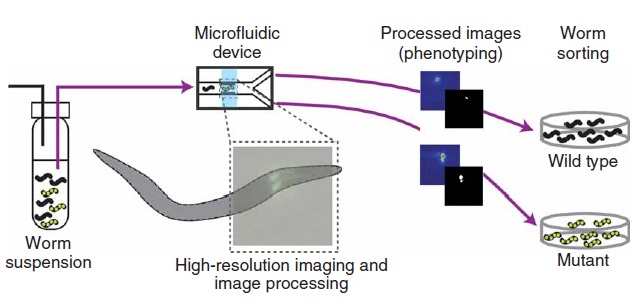
The microfluidic chip is manufactured by conventional soft lithography methods. It is made up of two layers, one for flow regulation and one for temperature control, divided by a 20 µm thick PDMS membrane (Fig. 1.3). The layers are bonded together and to the cover glass via oxygen plasma treatment. The microfluidic device is characterized by six salient features:
1) A built-in system to automatically regulate the loading of nematodes inside the chip, ensuring that just one worm at a time is present inside the imaging chamber.
2) A positioning system which automatically place with high precision each animal in the same location.
3) A temperature control system which provide the cooling needed for the immobilization of the sample.
4) Full compatibility of the set-up with any standard microscopy equipment.
5) Low cost and relatively ease of manufacturing.
6) High throughput and sorting efficiency.
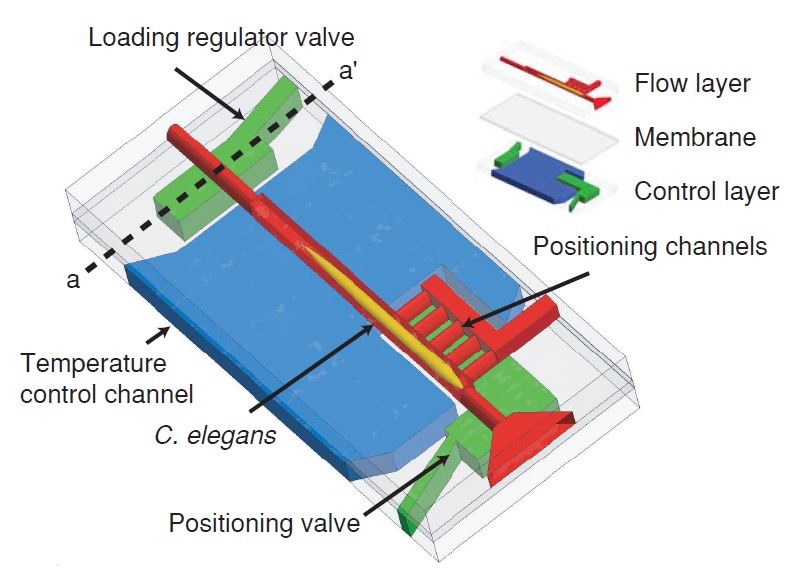
In order to load a worm into the chip, both outlet channels are closed while the side positioning channels remain open. The flow is then driven into channels by a constant pressure source. Furthermore, a system of loading regulator ensure that only one worm at a time is present in the area of the chip designated for imaging. This is possible because when an animal is present in the imaging chamber, the flow resistance of the chamber is increased as a result. Thereby, the flow rate – and hence the pressure – lowers on a second animal at the sample-loading regulator, which is located at the entrance of the imaging chamber. Consequently, this pressure drop makes impossible for the system to push the second worm inside. Then, as the first worm is released, this constrain is lifted and the pressure raises back to a point where the system is able to push inside the successive worm. In order to achieve precise and reproducible positioning of the warm inside the imaging chamber, the pressure difference between the positioning channels and the entrance of the main channel is exploited. Indeed, once the animal is positioned inside the detecting zone, the hydrodynamic resistance of the positioning channels self-equalizes and thereby the pressure is equally distributed on the body of the worm, preventing too high mechanical stress on the animal. This high-precision placing feature of the system allows to minimize the travel of the motorized stage of the microscope to locate the worm and makes the whole process less time-consuming as a result. Once placed in the imaging area, nematodes are cooled down to ~ 4 °C in order to immobilize them. This procedure does not employ any anesthetic and thus does not have any potential side effects on the sample due to drugs use. Furthermore, it has been proven to be as effective as immobilization by sodium azide. Finally, using microfluidic allows this setup to be easy and cheap to replicate as well as fully compatible with any standard microscopy setup.
2. Carbon dioxide immobilization of C. elegans on microfluidic chip
T. V. Chokshi et al. (2009) developed a technique for C. elegans immobilization which take advantage of a microfluidic chip with a built-in immobilization chamber where the worm is trapped by creating a CO2 micro-environment. Indeed, carbon dioxide is able to inhibit nematode’s movement for long period of time (up to 2 hours) without causing any permanent damage to the worm, which indeed can recover after a few seconds after immobilization. Moreover, this method has been proved to be suitable for worm of different age groups (L4’s to adults). The device employed is a two layer PDMS microfluidic chip (Fig. 2.1): one layer, called “behavior” module, is deputed to the revitalization of the animal after immobilization, while the other one is where the worm gets immobilized and thus is named immobilization module. The behavior module consists in a saw-shaped microchannel which forces the worm to move in a sinusoidal pattern so that its locomotion can be further analyzed. The immobilization layer contains the chamber where the carbon dioxide micro-environment is created in order to immobilize the worm, so that imaging can be performed. Finally, the two layers are separated by a 30 µm-thick PDMS membrane. The CO2 micro-environment is obtained by letting flow pure carbon dioxide through the control layer which diffuses through the membrane into the flow layer. As well know, PDMS is highly permeable to non-polar gases, hence carbon dioxide is able to replace the air in the immobilization chamber quite fast. Moreover, the membrane is thick enough to be able to sustain the gas pressures (10 psi) without noticeable deflections.
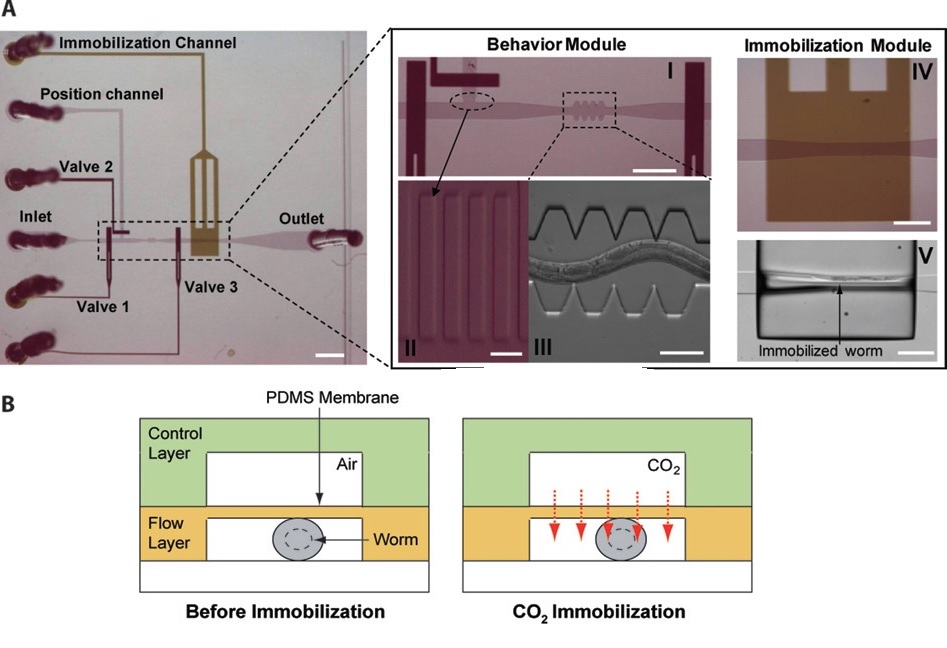
Worms are loaded into the main flow channel of the chip and manipulated by activating the integrated microfluidic valves (valves 1, 2 and 3 in Fig. 2.1) via the control channel. A separate channel (the “position” channel) perpendicular to the main loading channel is used to position the worm inside the behavior and immobilization modules. In order to prevent the worm from entering the position channel, some PDMS pillars (Fig. 2.1, II) are fabricated at its intersection with the loading channel. This technique can achieve long period of immobilization (up to 2 hours), thus allowing longer period for microscope observation. Moreover, the lack of oxygen in the immobilization chamber reduce photobleaching of fluorescent markers, making the CO2 method particularly appealing for long-term fluorescence imaging experiments such as time-lapse imaging of cell development.
3. Microfluidic array of clamps for C. elegans trapping
S. Elizabeth Hulme et al. (2007) designed a microfluidic device capable of mechanically trapping large (>100) numbers of C. elegans at a once. The procedure relies on the implementation in a microfluidic chip of wedge-shaped channels (Fig. 3.1), or “clamps”, which are able to trap the worm and thereby physically inhibit its movements. In particular, the width of the microchannel narrows gradually, lowering from 100 µm to 10 µm, over a length of 5 mm (Fig. 3.1, below)
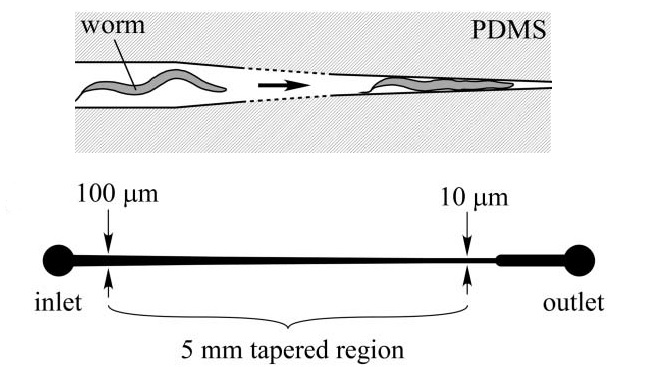
The device itself, consists of a network of distribution channel which supply an array of these clamps via a constant-pressure flow that drives the animals. As the immobilization takes place without drugs treatment, or any other potentially hurtful measures, this method does not disturb the natural biochemical state of the worm. Moreover, by reversing the direction of the flow, worms are released from the traps and repeated sampling of the same population is therefore possible. A constant pressure difference between the inlet and the outlet of the chip is required in order to drive and trap the worms into the clamps. It is important to underline that a constant pressure and not a constant flow is needed in this case, as the unbounded increase in pressure that would result from a constant flow regime could cause significant mechanical damage to the immobilized worms. Fig. 3.2 shows a schematic of the microfluidic device, which is basically made of an array of individual worm clamps. Channels are designed to tape gradually so worms of different sizes can be accommodated as, even in a isogenetic population, noticeable size difference between the individuals can be found. Therefore, the system can be also employed as analytic device to measure the size distribution of a given population of worms. Moreover, bifurcation has been chosen over higher order branching topologies to avoid any bias in worms’ distribution inside the chip. Finally, as a trapped worm increase the fluidic resistance of a given channel, when another worm arrives at the same junction it will not follow the same path; thus the device is designed to load a given clamp with only one worm. It is important to notice though, that no control is provided over the orientation of the worm when immobilized, i.e. if it gets trapped head-first or tail-first.
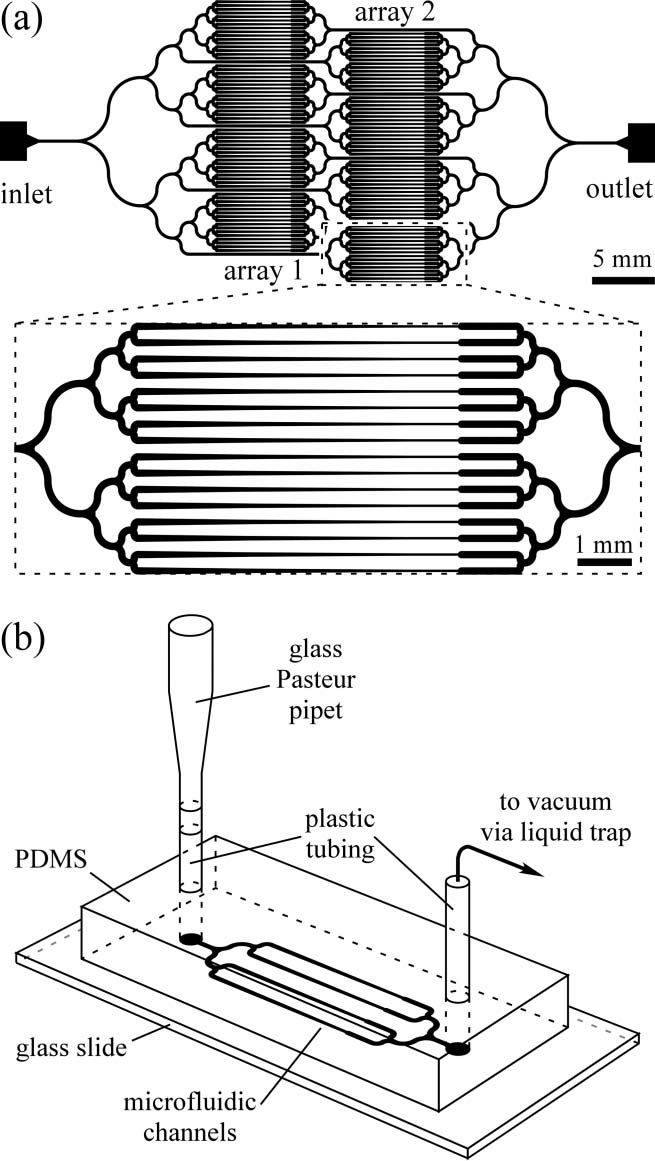
To wrap up, this technique allows, in quite a small period of time (~15 min), to reversibly immobilize a large number of nematodes without the means of any chemical compound. This can help researcher to save a lot of time during their experiment and ensure a high throughput during all observation time. Moreover, the use of a microfluidic device leaves the possibility of further improvements in the number of nematodes that can be trapped and also of implementing new feature adding other microfluidic components, e.g. for drug injection. Finally, as the immobilization device creates an ordered array of trapped worms, it should be possible, in principle, to automate the acquisition of data using a motorized microscope stage.
4. Compressive immobilization of C. elegans on microfluidic chip
This technique has been developed by Trushal V. Chokshi (T. V. Chokshi et al., 2009) and it is a variation of the carbon dioxide method described before (see §2). Indeed, the device design is almost identical – a two layer PDMS chip – with the only difference lying on the membrane that divides the two layers. Indeed, this time the immobilization of the animal is achieved mechanically: a high pressure (25 psi) air flow causes the membrane to deflect on the worm compressing it and, as a result, inhibiting its movements (Fig. 4.1).
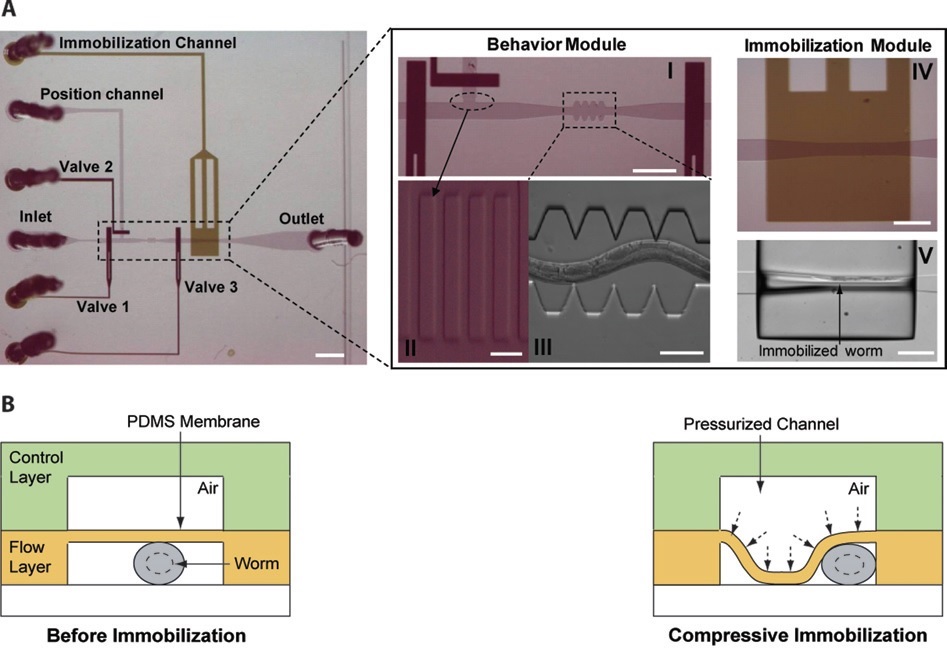
In order to maximize the effect of the immobilization a few changes are made to make the membrane more deformable. First of all, a 20:1 PDMS/curing agent mixing ratio is used rather than the conventional 10:1; this makes the PDMS elastic modulus decrease by a factor of two. Moreover, the width of the microchannel in the immobilization module is made wider (110 µm) than the width of the saw-shape micro- channel (90 µm) to allow large membrane deflections. The advantage of this method lies on the ease of manufacturing the microfluidic device and its further implementation. Moreover, this technique is suitable for animals of different ages and can be applied to large numbers of worms in a serial manner in order to increase the throughput. Finally, a shortcoming of this method is the rather short (minutes) immobilization time achievable compared to other techniques.
5. C. elegans immobilization in chip-gel hybrid microfluidic device
The methods described before are very efficient for morphological and phenotypes investigation, nevertheless present some shortcomings. Indeed, either they do not allow longitudinal studies of physiologically active processes (e.g. cooling method, see §1) or induce shifts on the anatomical features of the worm due to the compression employed to immobilize them (e.g., clamps and compressive method, see §3 and §4. ). Jan Krajniak and Hang Lu (2010) addressed these issues designing a hybrid microfluidic chip-gel system which allows to perform long-term high-resolution imaging of specific development processes at physiological conditions. In particular this device is able to culture, immobilize and image the nematodes all in a once. Reversible immobilization of C. elegans is achieved by using a commercially available bio-compatible polymer: Pluronic F127 (PF127). This polymer, in fact, is capable of reversible sol-gel transition which can be thermally-induced by a small temperature shift (~2 °C). In particular, when the PF127 is in its gel state (gelification) its viscosity increases at a level that inhibit any movements of the animal. The microfluidic device employed is a PDMS-based two layer chip (Fig. 5.1), where the two layers are separated by a thin PDMS membrane.
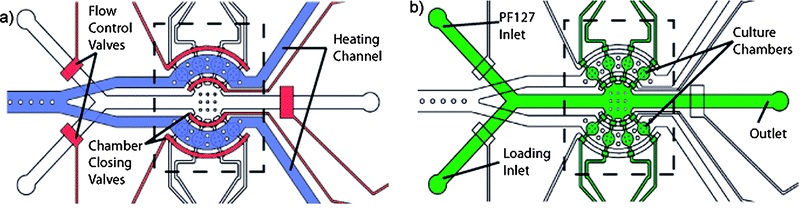
One module is deputed to load and flow control, whereas the other one is exploited for temperature control. Both channels have a thickness of 3 mm, while the membrane between them is 30 µm. The two layers of the chip are bonded together with a conventional oxygen plasma treatment. A key feature of this device is that worms are cultured within the device, as the latter is capable of providing both nutrient and gas exchange to nematodes inside its 8 chambers (Fig. 5.2).
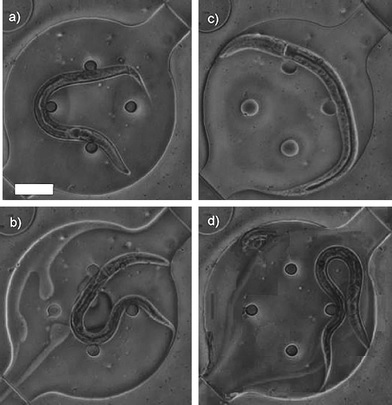
Furthermore, these chambers are used to keep animals separated during all investigation time, hence ensuring that development can be followed on a single-animal basis. The flow layer contains all flow inlets, which are used to load the worms and to supply the chamber with the PF127 solution, and the individual culture chambers. Furthermore, pneumatic valves are used in order to prevent escape of nematodes from the culture chambers. The second layer, the temperature control module, it used to produce small ( few °C) temperature changes on the device to trigger the PF127 transition. Precise temperature control is achieved by regulating the flow rate of the heating fluid and maintaining the heating fluid source temperature. As been said, the immobilization mechanism employed relies on a solution of PF127 which is lead into the culture chamber and goes under a reversible sol-gel transition triggered by thermal shifts. The immobilization thus take place anywhere in the chamber with no dependence on the worm placement, and with minimal environmental changes. In conclusion, this methods is able to immobilize up to 8 worms at a time for long time without interfering its biological functions, furthermore the immobilization protocol is fully reversible for a undefined number of times.
Conclusions
In this short review a few of the most notable microfluidic methods to achieve C. elegans immobilization have been presented. In particular, the quite obvious advantages of exploiting microfluidics for this goal have been underlined. Indeed, conventional immobilization methods, which rely mainly on glue or anesthetics drugs, have been proven to be time-consuming, low-throughput and also potentially hurtful for the sample compared to microfluidics alternatives. The obvious question arising now is: which microfluidic technique should I choose?
There is not a certain answer to this question, as which technique is the best for you strongly depends on your requirements and needs. Moreover, each of these methods ensure very good results in terms of C. elegans immobilization. Anyways, among them the Chokshi’s method provides the best comprise in terms of manufacturing difficulties over performance. The microfluidic device employed is, in fact, rather simple to realize as it is a two layer PDMS chip with a simple array of channels, in addition the same design can be used for two immobilization techniques (compressive and CO2) if either short (minutes) or long (hours) immobilization periods are required by the researcher. Furthermore, this method can be applied to worms of different age groups and allow fully recover from immobilization of the animal.
References
Aufderheide, K. J. (2008). An overview of techniques for immobilizing and viewing living cells. Micron 39, 71–76
Brenner, S. (1974). “The Genetics of Caenorhabditis elegans”. Genetics 77 (1): 71–94.
Kim E., Sun L., Gabel C. V., Fang-Yeng C. (2013). Long-term imaging of Caenorhabditis elegans using nanoparticle-mediated immobilization. PLoS ONE 8(1): e53419
San-Miguel A. and Lu H. , Microfluidics as a tool for C. elegans research*(September 24, 2013), WormBook, ed. The C. elegans Research Community, WormBook, doi/10.1895/wormbook.1.7.1.
Hulme S.E., Shevkoplyas S.S., Apfeld, J., Fontana W., and Whitesides G.M. (2007). A microfabricated array of clamps for immobilizing and imaging C. elegans. Lab Chip7, 1515-1523.
Chokshi T. V., Ben-Yakar A., and Chronis N. (2009). CO2 and compressive immobilization of C. elegans on-chip. Lab Chip 9, 151-157.
Chung K., Crane M. M., and Lu H. (2008). Automated on-chip rapid microscopy, phenotyping and sorting of C. elegans. Nat. Methods 5, 637-643.