Microtubules (MT) are involved in multiple cellular processes, including mitosis, cell transport, morphogenesis and motility. Two populations of microtubules have been described: cold labile and cold stable [1]. Both populations can be present in the same cell type, with different molecular composition and different cellular distribution. For instance, pulmonary endothelia contain both populations of microtubules [2].
This scientific note focuses on mechanisms protecting MTs from depolymerization by cold. The biological significance of cold stability from an evolutionary point of view is also discussed.
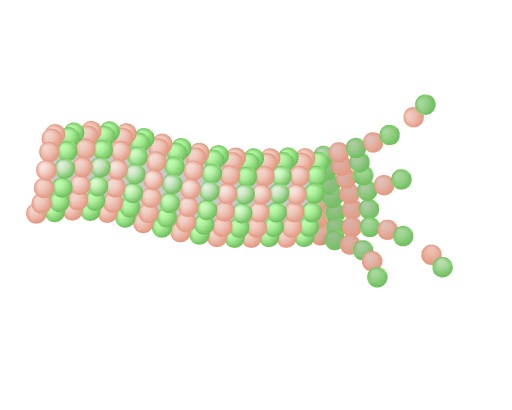
Ultra fast temperature shift device for in vitro experiments under microscopy
Cold stable microtubules
Mechanisms protecting cold stable microtubules
Some families of MAPs (Microtubule Associated Proteins) have been identified as central players in microtubule stabilization which renders them resistant to cold treatment. Initial studies on MAPs and cold resistance were performed in poikilotherm and cold adapted species, where cold protecting mechanisms are essential. To address the question of what renders microtubules cold stable in these species, in vitro assays for tubulin polymerization with MAPs purified from antarctic fishes were performed. At 0-5°C, addition of antarctic-fish MAPs to tubulin induced microtubule assembly. Bovine MAPs added to antarctic fish tubulin would still induce assembly at 2-5°C. On the contrary, antarctic MAPs added to bovine tubulin depolymerized at 0°C (while they were able to polymerize at 33°C). These studies led to the conclusion that antarctic fish MAPs don’t possess specific properties to stabilize microtubules at cold temperatures. Tubulin itself is resistant to cold depolymerization in cold adapted species through structural changes, mainly due to variations in amino acid composition [13,14,15]. Post-translational modifications of tubulin have been suggested to be responsible of the cold resistance properties (see below).
Nevertheless, further studies showed that MAPS can play a protecting role as well. Amongst the first MAPS identified with this property were the STOPs (Stable Tubule Only Proteins). They were initially found in the mammalian brain, associated to cold stable microtubules, in vivo and in vitro [9,10]. STOP proteins were later identified as a family of MAPS present in multiple tissues, from neurons to muscle, re-named as the MAP6 family. MAP6 are calmoduline regulated proteins, and this explains why cold resistant MT are sensitive to calmoduline. MAP6 proteins contain a domain which is responsible of cold resistance (Mc), while a second domain (Mn) is responsible of cold and nocodazole resistance [11].
One intriguing fact is how cold resistant microtubules are extended across the tree of life while the MAP6 family exists only in vertebrates, since identification of additional MAPs conferring resistance to cold was largely missing. Dacheux et al. [11] identified SAXO proteins as MAP6-like proteins specific to cilia and cilia-like structures, present in ciliated and flagellated organisms (including non-vertebrate species like Trypanosoma). SAXO MAPs are as well present in cilia and cilia-like structures in mammalian cells, like the human FAM154A (hSAXO1) [12]. Primary cilia are present in most human cell types, while motile cilia are present in some specific cell types (like sperm or tracheal epithelium). The cytoskeleton structure in both type of cilia is essential for their function, and defects in microtubule function and stability can lead to disease.
The importance of PTM on microtubule stability is well known. The effect of PTM on cold-resistance of microtubules has been largely investigated. Phosphorylation of microtubule associated proteins was suggested to play a role on cold resistance [8]. Additionally, acetylated and polyglutamylated microtubules were shown to be more resistant to cold, despite longer exposures to cold still made them depolymerize [4]. On the contrary, tyrosination is a PTM linked to cold-labile microtubules. As an example, two populations of microtubules were identified in the auditory sensory epithelium of the gerbil in a cell-type specific manner: cold sensitive microtubules in sensory cells, with tyrosinated tubulin, and cold-resistant microtubules in supporting cells with different PTMs. In this sensory epithelium, cold-sensitivity is linked to PTMs and this is important for cell function related to hearing [5].
Microtubule cold-protection mechanisms exist in poikilotherm and cold adapted species. However, it remains an open question which is, in vertebrate (and other) species, the physiological role of cold-resistant microtubules. In endothermic species, MAP6 has been described as a potential temperature sensor with a role in sensing body temperature decrease. A cold-resistant population of microtubules could help to maintain basal body functions under abnormal hypothermic conditions [17]. Mice lacking MAP6 show severe synaptic defects and abnormal behavior. This highlights the importance of microtubule stability for synaptic plasticity [16].
Tools for live imaging of microtubule response to cold have been lacking. The temperature controller CherryTemp allows to depolymerize and repolymerize microtubules in seconds, enabling to visualize subpopulations of microtubules and nucleation dynamics.
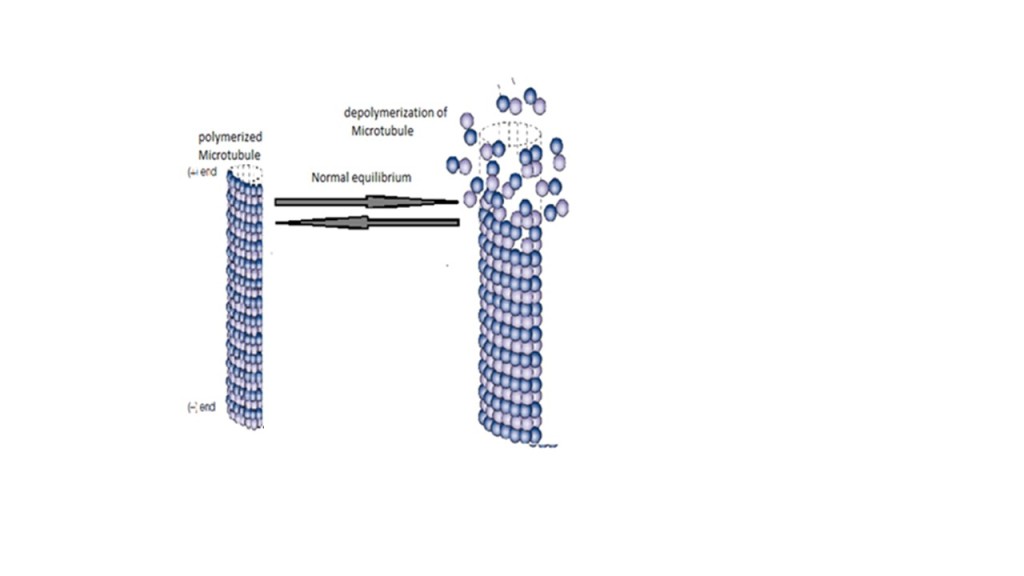
References
- [1] Jones DH. et al. Cold stable microtubules in brain studied in fractions and slices. J Neurocytol (1980) https://www.ncbi.nlm.nih.gov/pubmed/7441301
- [2] Ochoa C.D. et al. Cold exposure reveals two populations of microtubules in pulmonary Endothelia. Am J Physiol Lung Cell Mol Physiol (2010) https://www.ncbi.nlm.nih.gov/pubmed/20971804
- [3] Job D. et al. Rapid disassembly of cold-stable microtubules by calmodulin, PNAS (1981) https://www.ncbi.nlm.nih.gov/pubmed/6946418
- [4] Quinones GB et al. The posttranslational modification of tubulin undergoes a switch from detyrosination to acetylation as epithelial cells become polarized. Molecular Biology of the Cell (2011) https://www.ncbi.nlm.nih.gov/pubmed/21307336
- [5] Bane BC et al. Microtubule cold stability in supporting cells of the gerbil auditory sensory epithelium: correlation with tubulin post-translational modifications. Cell Tissue Res. (2002) https://www.ncbi.nlm.nih.gov/pubmed/11810314
- [6] Pirollet F. et al. Purification and characterization of sheep brain cold-stable microtubules. PNAS (1983) https://www.ncbi.nlm.nih.gov/pubmed/6572919
- [7] Job D. et al. Recycling of cold-stable microtubules: evidence that cold stability is due to substoichiometric polymer blocks. Biochemistry (1982) https://www.ncbi.nlm.nih.gov/pubmed/7066303
- [8] Margolis RL. et al. Characterization of rat brain crude extract microtubule assembly: correlation of cold stability with the phosphorylation state of a microtubule-associated 64K protein. Biochemistry (1981). https://www.ncbi.nlm.nih.gov/pubmed/7284335
- [9] Margolis RL et al. Purification and assay of cold-stable microtubules and STOP protein. Methods Enzymol (1986) https://www.ncbi.nlm.nih.gov/pubmed/3821559
- [10] Margolis RL. et al. Specific association of STOP protein with microtubules in vitro and with stable microtubules in mitotic spindles of cultured cells. EMBO J. (1990) https://www.ncbi.nlm.nih.gov/pubmed/2249667
- [11] Dacheux D. et al. A MAP6-related protein is present in protozoa and is involved in flagellum motility.Plos One (2012)
- [12] Dacheux D. et al. Human FAM154A (SAXO1) is a microtubule-stabilizing protein specific to cilia and related structures Journal of Cell Science (2015)
- [13] Detrich HW et al. Heterogeneity and structure of brain tubulins from cold-adapted Antarctic fishes. Comparison to brain tubulins from a temperate fish and a mammal. J. Biol Chem (1986) https://www.ncbi.nlm.nih.gov/pubmed/3733739
- [14] Detrich HW et al. Antarctic fish tubulins: heterogeneity, structure, amino acid compositions and charge. Comp Biochem Physiol B (1988) https://www.ncbi.nlm.nih.gov/pubmed/3180738
- [15] Detrich HW et al. Cold adaptation of microtubule assembly and dynamics. Structural interpretation of primary sequence changes present in the alpha- and beta-tubulins of Antarctic fishes. J Biol Chem (2000) https://www.ncbi.nlm.nih.gov/pubmed/10956651
- [16] Andrieux A. et al. The suppression of brain cold-stable microtubules in mice induces synaptic defects associated with neuroleptic-sensitive behavioral disorders. Genes and Dev (2002)
- [17] Delphin C. et al. MAP6-F Is a Temperature Sensor That Directly Binds to and Protects Microtubules from Cold-induced Depolymerization. The Journal of Biological Chemistry (2012)
- [18] Cottam DM. et al. Non-centrosomal microtubule-organising centres in cold-treated cultured Drosophila cells. Cell Motil Cytoskeleton (2006) https://www.ncbi.nlm.nih.gov/pubmed/16385467
- [19] Hayward D. and Wakefield JC. Chromatin-mediated microtubule nucleation in Drosophila syncytial embryos. Commun Integr Biol (2014). https://www.ncbi.nlm.nih.gov/pubmed/25053984